Quantum logic spectroscopy
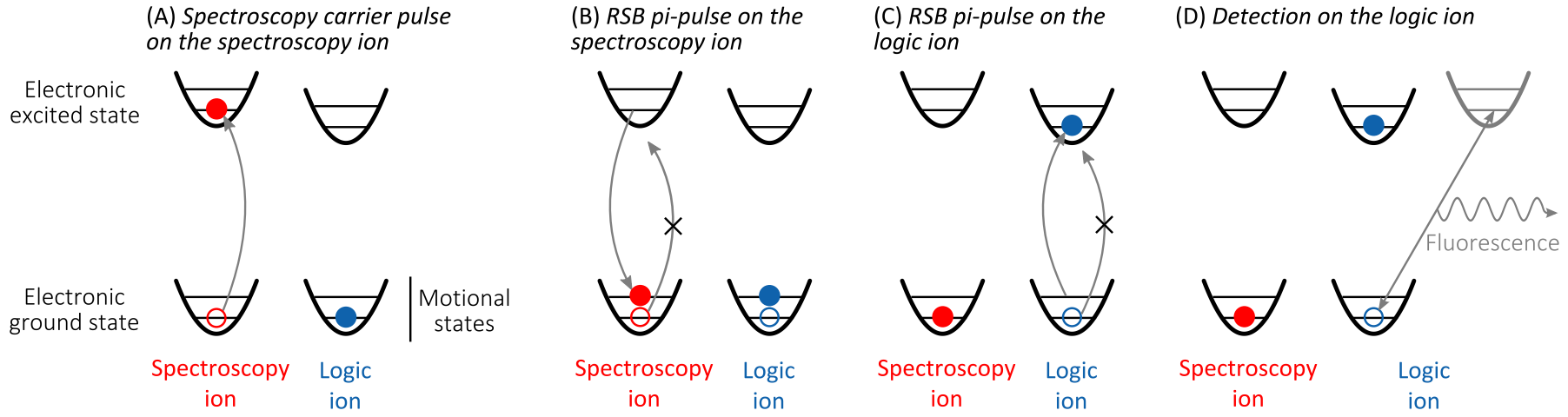
Quantum logic spectroscopy is a tool that makes it possible to read out the electronic state of an ion which does not possess a suitable transition for electron shelving, i.e. detection, on its own. This ion is referred to as the spectroscopy ion and could be, for instance, the Al+ clock ion in the quantum logic clock, a MgH+ molecular ion in the molecular spectroscopy experiment or a highly charged ion. The idea is to transfer the internal electronic state of the spectroscopy ion to another ion being trapped in the same harmonic potential. This second ion, the logic ion, has to have a broad and accessible transition for detection. As a logic ion we use Ca+, Mg+ or Be+, all of which have a suitable transition for coherent manipulations with resolved sidebands as well as a broad cycling transition for fluorescence detection. The motion of the two co-trapped ions is coupled via the Coulomb interaction and this coupling is ultimately used to transfer information from the electronic state of the spectroscopy ion to the logic ion where it can then be detected.
Quantum logic spectroscopy consists of a sequence of three single pulses and the detection. These four steps are now explained in more detail. At the beginning of each sequence both the spectroscopy and the logic ion have to be prepared in their electronic and motional ground states. The steps are schematically depicted in the figure above.
(A) Spectroscopy pulse on the spectroscopy ion. The spectroscopy interrogation is done by applying a carrier pulse on the clock ion. After the spectroscopy pulse the spectroscopy ion can either be found in the excited electronic state or, if the excitation was not successful, e.g. due to detuning of the spectroscopy laser, its state remains unchanged. For each of the following steps these two possible outcomes are distinguished.
(B) RSB π-pulse on the spectroscopy ion. If the spectroscopy pulse excited the spectroscopy ion, the RSB pulse transfers the ion back into its electronic ground state but adds one motional quantum. Since the motional mode is common to the two Coulomb-coupled ions, the logic ion’s motion is excited, too. If the spectroscopy pulse in (A) was not successful the RSB pulse has no effect, since its energy is too low (by one quantum of motion) to reach any available excited state, and the electronic and motional state are left as they were at the end of (A).
(C) RSB π-pulse on the logic ion. If the spectroscopy pulse in (A) excited the spectroscopy ion, the shared motional mode now contains one quantum of motional energy. Therefore a RSB π-pulse on the logic ion has sufficient energy to transfer it to the excited electronic state while removing the motional quantum from the two-ion system. If the spectroscopy pulse in (A) did not drive the spectroscopy transition, the motional mode is in its ground state and thus the RSB pulse does not have enough energy to transfer the logic ion into its excited electronic state.In the latter case, the state of the logic and spectroscopy ions is still unaffected.
(D) Detection. Finally, the electronic state of the logic ion is probed by the electron-shelving technique, where many fluorescence photons are scattered on a broad transition if and only if the logic ion is in its ground state. If the initial spectroscopy pulse on the spectroscopy ion was successful the logic ion is now in the excited electronic state and therefore appears dark during detection. If the spectroscopy transition has not been driven in (A) the logic ion is now in the electronic ground state and the detection transition can be driven causing it to fluorescence and the logic ion appears to be bright.